- 1Department of Immunology, Institute for Biomedical Aging Research, University of Innsbruck, Innsbruck, Austria
- 2Institute of Hygiene & Medical Microbiology, Department of Hygiene, Microbiology and Public Health, Medical University Innsbruck, Innsbruck, Austria
The immune system is a tightly regulated network which allows the development of defense mechanisms against foreign antigens and tolerance toward self-antigens. Regulatory T cells (Treg) contribute to immune homeostasis by maintaining unresponsiveness to self-antigens and suppressing exaggerated immune responses. Dysregulation of any of these processes can lead to serious consequences. Classically, Treg cell functions have been described in CD4+ T cells, but other immune cells also harbour the capacity to modulate immune responses. Regulatory functions have been described for different CD8+ T cell subsets, as well as other T cells such as γδT cells or NKT cells. In this review we describe the diverse populations of Treg cells and their role in different scenarios. Special attention is paid to the aging process, which is characterized by an altered composition of immune cells. Treg cells can contribute to the development of various age-related diseases but they are poorly characterized in aged individuals. The huge diversity of cells that display immune modulatory functions and the lack of universal markers to identify Treg make the expanding field of Treg research complex and challenging. There are still many open questions that need to be answered to solve the enigma of regulatory T cells.
Introduction
Immunological self-tolerance is the unresponsiveness of the adaptive immune system to self-antigens in primary lymphoid organs and further control of the activation, expansion and survival of self-reactive T and B cells in the periphery (1). The acquisition of immune tolerance is essential to avoid fighting one´s own cells and molecules. During thymic maturation of T cells somatic recombination leads to the expression of a distinct T cell receptor (TCR) on each individual T cell, which enables each T cell to recognize a specific antigen. The entirety of all T cell specificities is referred to as the repertoire. Binding of TCRs to self-peptide-loaded MHC molecules (self-pMHC) in the thymus leads to positive selection, and in a next step T cells binding self-pMHC with high affinity are eliminated by negative selection. Thymocytes can escape this clonal deletion by TCR gene rearrangement, which eventually changes the TCR affinity for self-pMHC. This central tolerance is not absolute as not all self-antigens are expressed in the thymus and due to the imperfect efficiency of the selection process. As a consequence, depletion of self-reactive T cells can also occur in the periphery and many self-reactive T cells can enter a state of unresponsiveness called “anergy” (peripheral tolerance) (2).
Some decades ago it was shown that animals harbor self-reactive T cells but also T cells that were able to suppress the autoimmunity caused by these cells (3, 4). These suppressor cells were later called regulatory T (Treg) cells and the surface molecule CD25 was identified as the first marker for this CD4+ T cell subset (5). It was not until 2003 that the transcription factor Forkhead box P3 (Foxp3) was described as a specific Treg marker by Rudensky’s laboratory. They discovered that Foxp3 deficient mice developed a lethal autoimmune syndrome and lacked CD4+ CD25+ cells (6). However, not all suppressor/regulatory T cells express the Foxp3 transcription factor, and many of those other populations have been described even before the Foxp3 expressing T cells. In this review, we will focus mainly on Foxp3 expressing Treg, their mechanisms of suppression and their role in different contexts. Nevertheless, a short description of Foxp3- Treg cells is necessary to understand the complexity of the exquisitely regulated homeostasis of the immune system. Regulation of immune responses requires not only the control of autoreactive immune cells but also the termination of immune responses in order to avoid chronic activation of the immune system. It is important to understand how these mechanisms are regulated in order to modulate immune responses in different disease settings. For instance, we need to strengthen immune responses in chronic infection or cancer but, on the other hand, immune responses need to be dampened when there is unwanted immunological activity (e.g. in autoimmunity or graft rejection).
Subsets of Regulatory T Cells
Naturally Arising Foxp3+ CD4+ Treg
Classically defined Treg are a subset of Foxp3 expressing CD4+ cells which maintain peripheral tolerance by suppressing autoreactive CD4+ cells that have escaped from negative selection in the thymus (7). Naturally arising Foxp3+ CD4+ Treg cells develop mostly in the thymus (tTreg) and require a relatively strong TCR signal which results in Treg cells having a repertoire enriched for self-antigen recognition (8). In the periphery, Treg can be generated upon TCR stimulation of naïve CD4+ Foxp3- T cells in the presence of TGFβ and they are known as peripheral Treg (pTreg) (9). In vitro, Treg can also be produced from CD4+ Foxp3- cells by mimicking in vivo conditions for pTreg generation (iTreg) (10). In contrast to tTreg, pTreg are likely generated upon exposure to non-self-antigens like allergens, food and microbiota (11).
It is important to keep in mind that whereas in mice Foxp3 expression is limited to Treg, many human Foxp3+ T cells are more similar to conventional T cells (Tconv) than to Treg, and some activated non-suppressive Tconv express low levels of Foxp3. Miyara and colleagues defined three different human T cell populations based on the expression of Foxp3 and CD45RA: Foxp3low CD45RA+ as resting Treg; Foxp3high CD45RA- as activated/effector Treg, and Foxp3low CD45RA- as non-suppressive cytokine-producing non-Treg (12). Thus, it is fundamental to combine Foxp3 expression with other Treg markers (CD45RA, CD127 (IL-7R), CD25) in order to identify and analyze these cells in humans (13).
CD4+ Foxp3+ T cells can modulate immune reactions in a direct or indirect fashion. One of the most studied direct suppression mechanisms is the production of the anti-inflammatory cytokine IL-10, which can inhibit phagocyte function, antigen presentation, co-stimulatory molecule expression, T-cell proliferation, and impairs the production of IL-2 and IFNγ. Treg-produced IL-10 promotes tolerance in the intestinal mucosa and defects in IL-10 signaling trigger inflammatory bowel disease in mouse and human (14, 15). In contrast, IL-10 can stimulate NK cell activity, B cell activation and isotype switching (16). Transforming growth factor-beta 1 (TGFβ1) signaling is associated with the development, stability and function of Treg. TGFβ1 antagonizes negative selection in the thymus, supporting early Treg development (17). In the periphery, it is essential for the differentiation of Treg from naïve CD4+ Treg. TGFβ1 production by Treg and its autocrine signaling is required for Treg-mediated suppression, but several studies suggest that while it is not a major suppressor mechanism it might be needed under high inflammatory conditions (18). When Foxp3+ CD4+ Treg encounter effector T cells (Teff) and interact with them, one mechanism of suppression is the secretion of granzyme and perforin via exocytosis. By doing so, they can induce apoptosis in the target cells, e.g. in CD4+ CD25- effector cells (19, 20).
Treg are able to indirectly turn down immune reactions by disturbing the optimal environment for immune responses by interfering with IL-2 availability, ATP/AMP balance, and the interface between T cells and DC. IL-2 is known for inducing and promoting T cell proliferation, but it also is involved in termination of T cell responses (21), since mice deficient in IL-2 or IL-2R suffer from a lymphoproliferative syndrome (22, 23). This negative effect on T cell activation happens indirectly by promoting the activation of anergic Treg, which then in turn suppress other T cells (24). Upon activation of naïve T cells, IL-2 is produced, which induces phosphorylation of STAT5 promoting Foxp3, Tbet and GATA3 expression and thereby the generation of Treg, Th1, or Th2 cells, respectively. At the same time, production of IL-17A and Bcl-6 and thereby differentiation towards Th17 or Tfh cells is inhibited (25, 26). Interestingly, high concentrations of IL-2 favor differentiation of effector T cells (27), whereas low IL-2 levels facilitate the production of memory T cell (28). Treg can interfere with these processes by modulating the amount of available IL-2. They suppress production of IL-2 by effector cells in a contact dependent manner in vitro. It has also been suggested that Treg can sense the source of IL-2 and migrate to the zones of immune activation where they “steal” IL-2 from other T cells promoting their apoptosis. This model remains controversial regarding in vivo studies since the source of IL-2 needs to be clarified (18).
Murine CD4+ Treg express high levels of the two ectonucleotidases CD39 and CD73 which can convert ATP into non-toxic AMP and AMP into the immune suppressive adenosine, respectively. In humans, co-expression of these ectonucleases is a rare event and most Treg express only CD39 which means they need to encounter CD73+ cells in order to produce adenosine (29). Extracellular adenosine binds the A2AR receptor expressed by Treg increasing their frequency and promoting their immune modulatory function (30). In the presence of excessive inflammation and tissue damage, there is an increase of extracellular ATP, which is cytotoxic for many cell types. Extracellular ATP promotes death signaling through P2X7R engagement in sensitive Treg while some Treg survive and convert into effector Th17 cells upon exposure to extracellular ATP (31). An additional strategy of Treg to escape apoptosis and survive in such environments is the conversion of ATP into non-toxic metabolites, such as AMP. In addition, AMP can indirectly alter the expression of proinflammatory cytokines and promote the expression of inhibitory ones. This translates into a reduction of costimulatory molecules in DC (32), less activation of effector cells and a higher suppressive capacity of Treg (30).
Treg can disrupt the microenvironment in the immunological synapse provided by DC which is essential for T cell proliferation. In detail, Treg act by either reducing the limiting enzyme for glutathione (GSH) synthesis or by consuming extracellular cysteine which is needed for T cell cycle progression and DNA synthesis (33).
Treg are also capable of removing surface molecules from antigen presenting cells (APC) during the immunological synapse. They can engulf part of DC membranes containing pMHCII and co-stimulatory molecules which leads to abrogation of T cell priming (34). Moreover, the inhibitory molecule CTLA-4, which is constitutively expressed on Treg has been described to remove CD80/CD86 from the surface of antigen presenting cells during the immunological synapse (35).
Some Treg have developed specialized adaptations to their environment. As an example, VAT-Treg (visceral adipose tissue-Treg) express high levels of PPARγ in order to reduce insulin resistance associated with inflammation of fat tissue (36).
Non-Classical CD4+ Treg
As already mentioned above, there are other types of regulatory T cells that do not fit the phenotype of the classically defined CD4+ Foxp3+ Treg cells (Table 1). Many of them share the mechanisms of suppression of Treg described above, but some use different strategies.
Type 1 regulatory T cells (Tr1) are a population of CD4+ Foxp3- cells expressing high levels of the anti-inflammatory cytokine IL-10. They are generated in the mucosa-associated lymphoid tissue (MALT) when naïve CD4+ cells encounter IL-10 produced by APC. Tr1 cells control T cell responses in infection and autoimmunity and they have been shown to produce higher levels of IL-10 than Foxp3+ Treg (37). Another subset of adaptive Treg are the Th3 cells, which are a unique population of T helper cells induced by oral tolerance to non-self-antigens. Th3 cells produce high concentrations of TGFβ and moderate amounts of IL-10 (38). In contrast to Tr1 cells which do not express Foxp3, some Th3 cells are TGFβ-induced-Foxp3+ cells (51). Collison and colleagues described another inducible type of Treg (iTr35) which do not secrete IL-10 or TGFβ, but instead IL-35, an inhibitory member of the IL-12 pro-inflammatory family. This cytokine suppresses T cell responses and expands Treg by inducing the conversion of conventional T cells into suppressive Foxp3- regulatory T cells (iTr35). iTr35 cells are highly suppressive and stable in vivo, they are key mediators of infectious tolerance and can contribute to Treg-mediated tumor progression (39).
Similar to other components of the immune system, B cells are involved in the expansion and generation of Treg. In the periphery, naïve B cells can convert CD4+ CD25- cells into CD4+ CD25+ Foxp3- Treg (Treg-of-B cells) in a cell-cell contact dependent manner. These Treg-of-B cells express molecules characteristic for Treg, such as IL-10, TGFβ, CTLA-4, PD-1, LAG-3, GITR, ICOS, and OX40. They exert their suppressive function in vivo and in vitro in antigen-specific and antigen-independent manners, utilizing IL-10-mediated as well as other suppressive mechanisms (40).
CD8+ Regulatory T Cells
CD8+ T cells were first described to exert immunosuppressive functions more than 40 years ago, but the lack of specific markers and therefore the difficulties in isolating this population limited this area of research (52). Interest in CD8+ Treg cells increased again after the revival of the concept of T-cell-mediated immunosuppression in the mid-1990s and the study of CD4+ Treg cells. In the following years the immunosuppressive properties of CD8+ cells were independently characterized by several groups (53).
Foxp3 is preferentially expressed in CD4+ CD25+ cells in mice and it is barely detectable in CD8+ T cells. The expression of the transcription factor HELIOS or the surface marker CD122 or the lack of CD28 on the surface are used to identify CD8+ Treg in mice (46). In contrast, human CD4+ as well as CD8+ T cells are able to express Foxp3, but levels of this transcription factor are substantially higher in CD4+ compared to CD8+ cells (54). In contrast to CD4+ regulatory T cells, there is no reliable marker to define CD8+ Treg cells, but they are rather identified by their immunosuppressive function.
Firstly isolated from rats, CD8+ CD45Clow/- Treg cells suppress the proliferation of CD4+ T cells and their differentiation into a Th1 phenotype. They produce IL-4, IL-10, and IL-13 and express CTLA-4 and Foxp3 (45). Sorted human IFNγ+ IL‐10+ CD8+ CD45RClow/‐ Treg are more potent suppressor cells than the rest of the CD8+ CD45RClow/‐ Treg and blockage of IFNγ abrogated their suppressive activity in a model of allogenic cardiac transplantation (55).
Foxp3+ CD8+ cells are rarely detected in human blood but they are found in human tonsils where they express high levels of CTLA-4 and CD45RO but only little CD127 and CD69. Tonsillar Foxp3+ CD8+ Treg are CD25- and express pro-inflammatory cytokines like TNFα, IFNγ, and IL-17 (41). Foxp3 expressing CD8+ T cells have also been found in blood of HIV-infected individuals showing an activated (HLA-DR, Ki-67, and PD-1 expression) and senescent (CD57+ CD28-) phenotype (56). Several studies show that CD8+ Foxp3+ Treg can be induced under certain conditions. In vitro stimulation of peripheral blood mononuclear cells (PBMC) with anti-CD3 mAb has been shown to induce CD8+ CD25+ Foxp3+ T cells, which were able to suppress proliferative responses to Staphylococcal enterotoxin B (SEB), and that the inhibitory effect was partially depending on CCL-4, TNF, and IL-2 (43). CD8+ CD28- Foxp3+ cells can be generated in vitro after multiple rounds of stimulation of PBMC with allogenic or xenogenic APC. They are believed to tolerize APC by up-regulating the inhibitory receptors immunoglobulin-like transcript 3 (ILT-3) and 4 (ILT-4) and down-regulating costimulatory molecules such as CD58 and CD86 (42). Upon suboptimal TCR stimulation in the presence of IL-15, CD8+ CCR7+ T cells express Foxp3 (they become CD8+ CD45RA+ CCR7+ Foxp3+) and acquire immunosuppressive functions. They prevent CD4+ T cells from responding to TCR stimulation by directly interfering with the TCR signalling cascade and not by the usual suppression mechanisms mediated by IL-10, TGFβ, or CTLA-4 (44). In addition, these CD8+ Treg release exosomes carrying NADPH oxidase 2 (NOX2), which are taken up by CD4+ T cells and inhibit their proliferation in vivo and in vitro (57).
Most studies investigating non-Foxp3 expressing CD8+ Treg in humans describe them as CD8+ CD28- cells, although CD8+ CD28+ Treg can be generated in vitro. Mechanistically, CD8+ CD28- Treg act by i) influencing CD80/CD86 surface expression of DC leading to inhibition of CD4+ T cell responses, or ii) secretion of IFNγ and IL-6 cytokines or iii) secretion of the anti-inflammatory cytokine IL-10 (54). In addition, CD8+ CD28- Treg express high levels of the IL-2 receptor CD122 and this has been used as a marker for the characterization of these CD8+ CD28- Treg. On the other hand, a population of CD8+ CD28+ Treg cells expressing the chemokine receptor CXCR5 has been identified, which are capable of suppressing B cell responses and antibody production by inhibiting follicular helper T (Tfh) cell-mediated B cell differentiation (58). They also exert strong antitumor activity and their presence is associated with favorable prognosis in follicular lymphoma patients (59).
In mice and humans, CD8+ Treg have been described to preferentially recognize the non‐classical MHC class I molecules Qa‐1 (mouse) or HLA‐E (human) which are orthologous genes. These non‐classical MHC class I restricted populations have the property to recognize TCR, MHC or heat shock protein derived peptides (i.e. Qdm, HSP60sp) presented by Qa‐1 or HLA‐E (48). CD8+ Treg exert a cytotoxic effect against antigen−activated CD4+ T cells, and this function depends on the expression of the MHC−Ib molecule Qa−1 in mice (54).
Whereas CD8+ CD45RO+ CCR7+ T cells are found in blood and have no suppressive function, CD8+ CD45RO+ CCR7+ IL-10+ suppressive cells are found intratumorally in ovarian cancer patients and they are believed to be induced by plasmacytoid DC (60).
In 2014, a novel population of CD8+ Treg characterized by the expression of HLA-DR was identified in human peripheral blood and umbilical cord blood. CD8+ HLA-DR+ cells suppress in a cell-to cell contact dependent manner, which involves CTLA-4 (47). Within the CD8+ HLA-DR+ Treg cells the CD28+ subpopulation shows higher suppressive capacity compared to their CD28- counterparts and also expresses higher levels of the checkpoint inhibitory molecules CTLA-4, TIM-3, PD-1 and LAG-3 (61). Similarities have been found between CD8+ HLA-DR+ and CD4+ Foxp3+ Treg with regards to the expression of TIGIT, the chemokine receptors CCR4 and CCR5, the low expression of IL-7R (CD127) and a memory and effector-like phenotype. In addition, after polyclonal TCR stimulation, CD8+ HLA-DR+ Treg cells increase IFNγ and TNFα expression suggesting that they are not exhausted cells despite the fact that they express PD-1 (62).
CD4−CD8− Regulatory T Cells
Gamma-delta T cells (γδT) are the first T cells to develop in the thymus upon gene rearrangement which generates different TCR chains (γδ) than the more abundant αβT cells during fetal ontogeny. In contrast to αβT cells, γδT cells do not undergo thymic TCR selection (63). γδT cells represent a small T cell population (3–5% in human peripheral blood) and are able to interact with different immune cell types such as other T cells, B cells, DC, NK cells, monocytes/macrophages, and granulocytes. In some cases, they exert an anti-inflammatory effect. These regulatory γδT cells have been studied in different contexts and are associated with immunosuppression in pregnancy, inflammation, allergy and cancer. Similarly to αβT cells, γδT cells produce TGFβ and IL-10 together with variable expression of the transcription factor Foxp3 (49).
Natural killer T (NKT) cells are a special subset of T cells that co-express NK cell surface receptors (NK1.1/CD161) with the semi-invariant T-cell receptors (TCR), which consist of an invariant TCR α chain paired to a limited number of TCR β chains. Like all T cells, they are generated in the thymus, but most of the NKT cells do not express CD4 or CD8 on their surface (64). Upon activation, NKT cells produce large amounts of Th1 (including IFNγ and TNFα) and Th2 (IL-4, IL-10, and IL-13) cytokines enabling them to act as powerful regulators of the immune system (50). Under certain conditions, NKT cells can exert potent suppressor functions by shifting from Th1 to Th2 responses both in human and mouse. Their main target cells of suppression are tumor cells, pathogen-activated T cells and APCs (65).
Roles of Treg in Different Scenarios
Treg constitute a group of phenotypically distinct subsets that can reside in lymphoid and in non-lymphoid organs where they exert diverse functions. The main non-lymphoid tissues where Treg can be found are the visceral adipose tissue (VAT), the intestine, skin and muscle. In these four tissues Treg are important regulators of inflammation and fibrosis and contribute to tissue repair (66). As already mentioned, Treg participate in numerous processes in which they adapt to the environment in order to eventually maintain tissue homeostasis. Some situations such as pregnancy, organ transplants or the common presence of bacteria in the intestine, require tolerance against foreign antigens for which Treg activity is essential. If Treg activity is too low, there can be a failure in self-tolerance leading to the development of autoimmune diseases. On the other hand, it can be hypothesized that if Treg are over-active, they may favor the progression of neoplasic malignancies. During pregnancy, the allogenic nature of the fetus (harboring maternal and paternal antigens) requires tolerance from the mother’s immune system in order not to be rejected. Treg and other immune cells create a tolerogenic environment whose composition changes throughout gestation. At least five different Foxp3+ Treg subtypes have been identified during different stages of pregnancy. In addition, other minor Treg subsets such as CD4+ HLA-G+ Foxp3- (which inhibit NK, CD4+ and CD8+ T cells), Tr1, Th3, γδT cells, TIGIT+ T cells, and CD8+ Treg have been detected in the decidua and/or peripheral blood of pregnant women (67).
Barrier tissues are constantly exposed to dietary, environmental, and commensal microbiota antigens and therefore immune homeostasis and tolerance need to be ensured via Treg or Teff antigen-specific repertoires in these tissues (68). In intestinal tissues, this tolerance is achieved by the cooperation of different immune populations including Foxp3+ Treg and Tr1 cells. Dysregulated intestinal responses to dietary antigens or commensal microbiota frequently lead to immunological disorders in humans such as celiac disease, food allergy, and inflammatory bowel disease (69). Encounter with commensal microbiota generates pTreg rather than anti-microbial effector cells. These pTreg use site-specific TCRs different than the ones that facilitate tTreg development in the thymus, implying that many colonic Treg arise by means of antigen-specific driven pTreg development (70).
Several reports highlight Treg functional deficiencies in autoimmune diseases, but the underlying molecular mechanisms are still unknown. One of the major limitations of studying human autoimmunity is the lack of validated experimental assays and the discrepancies between in vitro and in vivo experiments (71). Also, there is little consensus for Treg identification (i.e. agreement on the markers used for their identification), which makes the comparison between different studies almost impossible (13). Treg dysfunction in autoimmune diseases can be grouped according to different factors (68): i) Genetic disease like germline mutations in the Foxp3 locus. The development of the severe immune dysregulation, polyendocrinopathy, enteropathy, and X-linked (IPEX) syndrome is due to point mutations and microdeletions in the Foxp3 gene that impair Treg function (72). ii) The abrogation of Treg promoting signals. The disruption of the IL-2/IL-2R pathway dysregulates thymic development and peripheral homeostasis of Treg. In a murine model of Type 1 diabetes, pancreatic Treg die showing a decreased expression of the IL-2R CD25 and of the anti-apoptotic protein Bcl-2 (73). iii) The presence of Treg destabilizing factors. Overexpression of IL-6 and TNFα can interfere with Foxp3 expression and consequently alter Treg/Teff balance. In the presence of TGFβ, IL-6 enhances RORγt expression, which induces Th17 generation via STAT-3, and represses at the same time Foxp3 expression. In the case of rheumatoid arthritis, high IL-6 levels are related to a preferential development of Th17 cells over Treg in the periphery (74, 75).
In addition to maintaining immune homeostasis in the lymphoid tissues, Treg are recognized as regulators of non-immunological processes. Treg are present in healthy tissues and upon tissue injury, they promote tissue regeneration in an amphiregulin-dependent manner (76). In a model of influenza virus infection, Treg-induced tissue repair is triggered in response to the inflammatory mediators IL-18 and IL-33, but not by TCR signaling, which is required for their suppressive function (77).
Increased immune suppression contributes to cancer onset and tumors promote the generation of an environment that allows tumor cells to escape from immune responses. Cancer cells develop immunosuppressive mechanisms such as expression of anti-inflammatory mediators and recruitment of suppressive leukocytes, such as Treg, myeloid derived suppressor cells (MDSC), tolerogenic DC and tumor-associated macrophages (TAMs) (68). In recent years it has been found that many tumors are enriched in Treg cells, indeed, a high Treg/Teff ratio correlates with poor prognosis (78). Treg may facilitate cancer progression via suppression of effector cells that otherwise would attack the tumor. Turnis and colleagues showed enrichment of IL-35-secreting Treg cells in tumors and demonstrated that Treg-derived IL-35 promotes T cell exhaustion in the tumor microenvironment (79). In fact, different tumor models benefit from Treg depletion leading to an increased anti-tumor response (80–82), but not all tumors benefit from the presence of Treg cells. In addition, CD4+ CD25+ Treg collaborate with CD8+ CD28- Treg cells within different tumors so that the immunosuppressive activity of these tumor infiltrating Treg cells may be predominant (83). In multiple myeloma patients, CD8+ CD57+ lymphocytes show an activated phenotype (HLA-DR+ and Fas+) and can inhibit the suppressive effect of Treg as well as antibody production (84). However, the effect of Treg depends on the tumor site, molecular subtype and tumor stage (85). Interestingly, Foxp3+ tumor infiltrating Treg were associated with better prognosis in colorectal cancer (86). These cells were later shown to not be fully suppressive and to display some inflammatory T cell features (87). In human follicular lymphoma, high amounts of intratumoral Treg were related to positive outcome (88, 89) whereas high levels of circulating Treg correlated with a negative prognosis (90). All these discrepancies indicate that the presence of intratumoral or circulating Treg may depend on the nature of the tumor, the tumor microenvironment itself and the functionality of the supposedly suppressive Treg.
Low responsiveness and reduced proliferation of virus-specific T cells during chronic viral infection is associated with the expansion of Treg. In acute and chronic murine retroviral infection models, depletion of Treg decreases viral load and restores the activity of virus-specific cytotoxic CD8+ T cells (91, 92). On the other hand, it has been found that the role of Treg depends on the disease stage in tuberculosis patients. Treg expand and delay immune responses in initial phases but they counter-regulate excessive inflammation later in the chronic phase (93). In a mouse model of chronic infection with Friend retrovirus (FV), vaccination with a calcium phosphate nanoparticle-adjuvant, which efficiently reactivated CD8+ T cells, in combination with a transient ablation of Treg enhances anti-viral immunity (94).
Germinal centers (GC) are transient structures in peripheral lymphoid organs where B cells develop and differentiate into antibody secreting plasma and memory B cells. Upon activation by follicular dendritic cells, B cells proliferate and interact with primed antigen-specific Tfh cells in order to be fully activated and differentiate into antibody-secreting plasmablasts (95). Recently, a new Treg subset has been described in GC called follicular regulatory T cells (Tfr). These Tfr cells control Tfh-driven GC responses preventing induction of autoreactive and foreign antigen-specific antibodies (96). They control IgG and IgE responses to vaccines, allergens and autoantigens, and have a critical immunoregulatory function before GC formation (97). The relevance of these Tfr in the control of antibody production has drawn the attention of several studies which investigate these cells as new targets for immunotherapy.
Treg Homeostasis and Function in Aging
The severity of many infections increases with age and many of the vaccines currently used are less effective in older compared to younger adults. This is due to changes that the immune system undergoes over time leading to dysregulation the adaptive and, to a lesser extent, innate mechanisms (Table 2). In old age, the capacity of APC to process and present antigens to T cells declines (98) and chemotaxis, cytokine production and signal transduction upon antigen recognition are impaired in these cells as well as in neutrophils (99, 100). In addition, B cells show reduced somatic hypermutation and class switch and the number of plasma cells is decreased which leads to lower antibody production (101). The T cell compartment is skewed towards effector/memory like T cells, shows a reduced TCR repertoire and also accumulates DNA damage in aged individuals (102). In this review, we aim to describe the processes relevant for Treg, but extensive reviews on general immunosenescence can be found elsewhere (103–105).
During aging, the thymus undergoes a gradual reduction in size and function together with changes in its architecture (106). The activity of key factors for thymus functionality is also modified. Less bone marrow progenitors reach the thymus, the flow of sex hormones is diminished, thymic epithelial cell (TEC) number decreases and there is an increase in adipose tissue (107). TEC are part of the structural environment necessary to support the normal differentiation of thymocytes. TEC-mediated thymic involution results in reduced cellularity capable of maintaining normal thymocyte differentiation (108). Despite the decline in thymic cellularity, no blockade of thymocyte differentiation is observed and thymic function is maintained proportional to the reduced size (109). Adipocytes are not only responsible for anatomical changes within the thymus but they actively contribute to thymic involution. They produce higher levels of negative factors for thymic maintenance (e.g. IL-6, sex hormones and steroids) and thereby transmit suppressive signals to TEC reducing thymopoiesis and cellularity (107). During aging, the overall endocrine profile changes with an extra-thymic reduction of sex hormones and growth hormones, and an increase in glucocorticoid levels (110). Estriol and chorionic gonadotropin positively affect Foxp3 expression and increase Treg frequency. Steroids and glucocorticoids can enlarge Treg populations in the periphery stimulating their function (111). In fact, Treg lacking the glucocorticoid receptor lose their suppressive function turning into Th1-like, IFNγ-producing cells in a murine colitis model (112).
In the periphery, the number of recent thymic emigrants (RTE) is decreased in aged mice and humans which implies a lower contribution of thymic cells to the pool of total T cells in the body (113–115). In mice, it has been observed that the production of Treg cells declines more and faster than conventional T cell production. This is attributed to the inhibitory effect of recirculating Treg cells on the differentiation of new Treg when the former revisit the thymus (116). This accumulation of antigen-specific Treg reduces clonal diversity which translates into a skewed aged Treg pool which can suppress only certain T cells while leaving the rest unaffected. This might favor some proinflammatory cells to remain active in aged hosts (111).
Aging and age-related diseases are associated with profound changes in epigenetic patterns. Treg are subject of epigenetic modifications that are altered during aging. It has been shown that hypomethylation of CpG sites upstream of the Foxp3 enhancer correlates with a higher suppressive function of Treg from aged mice (117).
In addition, pTreg differentiation also decreases with age. It has been observed that naive conventional T cells from aged mice differentiate less into pTreg in vivo and in vitro compared to their young counterparts (118). Despite less thymic and peripheral Treg differentiation, Treg accumulate with age, which could be explained by the loss of the pro-apoptotic protein Bim rendering these cells apoptosis-resistant compared to Treg from young individuals (119). Loss of Bim or overexpression of Bcl-2 lead to Treg accumulation, but at the same time to reduced suppressive capacity in a model of murine colitis (120). On the other hand, in vitro studies show that Treg from young and old adults have the same suppressive capacity (121). Others describe that old Treg are better suppressors than Treg from young adult mice due to a higher IL-10 production from the older ones (117). Despite many discrepancies among different studies, the overall data suggests that Treg function remains unchanged or is even enhanced in the elderly. Aged individuals are more prone to develop infections and neoplastic malignancies which would agree with enhanced Treg function, whereas they are also more susceptible to develop autoimmunity due to Treg dysfunction (122).
In the periphery, total T cell numbers remain unchanged with age maintaining an adequate pool of circulating T cells including Treg. In peripheral blood mononuclear cells (PBMC) from aged humans an increased proportion of activated Treg (Foxp3hi CD45RA-) and a low but detectable resting Treg population (Foxp3low CD45RA+) are present. Both populations show suppressive potential but the activated Treg die after exerting in vitro suppression (12). It has been observed that there is an accumulation of CD25low Foxp3+ Treg in old age and this correlates with lower IL-2 levels. The IL-2 receptor (IL-2R), consist of the subunits IL-2Rα (CD25), IL-2Rβ (CD122) and the common gamma chain (CD132), and is expressed on T and B cells, DC and steady state NK cells. CD25low Foxp3+ Treg upregulate the surface molecule CD122, which is also part of the IL-15 receptor, suggesting that IL-15 might support CD25low Treg in old age (123). Tr1 cells also accumulate in aged mice in an IL-6 dependent manner and are able to produce large amounts of IL-10 (37). Follow-up studies show that these IL-10 producing cells manifest a Tfh phenotype and that they are involved in immune suppression in old age (124). Van der Geest and colleagues studied different CD4+ T cell populations in healthy individuals and observed that the proportion of naive Treg cells declined with age whereas the memory Treg compartment and the memory Treg/Teff ratio increased. This accumulation of memory Treg in old age is associated with poor responses to influenza vaccination (125). Tfh cells from aged mice express high levels if IFNγ and IL-10 and an environmental increase in TGFβ levels in old mice favors the development of Treg (126). After vaccination in old age, impaired differentiation of Tfh cells followed by a suboptimal T cell priming is observed with a consequent poor GC B cell expansion. This is thought to be due to not only lower T cell activation but also to an accumulation of Treg which negatively affect the GC reaction (127). Along these lines, another study in aged patients showed that non-responder individuals to influenza vaccination have a higher frequency of Treg as well as a higher inflammatory status compared to responders (128). Depletion of Treg with anti-CD25 treatment prior to influenza infection protected against lethal viral challenge in aged mice (129). In another study, responders to influenza vaccination showed a higher frequency of Treg compared to non-responders, together with an elevated frequency of early differentiated CD4+ T cells and lower proportion of memory CD4+ cells (130). Actually, a detailed study in the context of inflammation showed that, upon secondary activation, memory Treg could not undergo pronounced recall expansion as conventional CD4+ T cells do (131). These findings emphasize the importance of the accumulation of memory Treg during aging and their role in the ineffectiveness of vaccination in the elderly.
Similar to CD4+ cells, the CD8+ T cell pool also loses naïve precursor cells but the contraction of the naïve CD8+ T compartment is greater than the one for CD4+ T cells (132). In human and mouse, naturally occurring CD8+ Foxp3+ Treg numbers increase with age (133, 134) whereas inducible CD8+ CD45RA+ CCR7+ human (135) and CD8+ CD44+ CD62L+ CCR7+ mouse (43) Treg cells decline in old age. The increase of CD8+ Foxp3+ CD28– Treg is consistent with the higher overall numbers of CD8+ CD28– T cells in aged individuals (136). Despite the fact that CD8+ HLA-DR+ Treg accumulate with age, the expression of CD28 in these cells remains unchanged. CD8+ HLA-DR+ Treg cells lose suppressive activity and decrease the production of checkpoint inhibitory molecules in aged individuals (61).
Together with CD4+ CD25+ Treg frequencies observed in the elderly, CD8+ Treg accumulation has been suggested to contribute to the decline in adaptive immune responses in the aging process (137). The capability of human CD8+ CCR7+ cells to differentiate into Foxp3-expressing cells negatively correlates with age (44). It has been shown that expression levels of Foxp3 and CD45RA in CD8+ CCR7+ Treg are lower in older individuals compared to young, and this may suggest a diminished suppressive capacity of these cells potentially contributing to the development of autoimmune diseases in the elderly (138). In contrast, the ability of aged naturally occurring CD8+ Treg to suppress the proliferation and cytokine production of effector CD4+ T cells remains similar between younger and elder individuals (137). Dysfunction of CD8+ and CD4+ Treg cells in old age could support age-associated subclinical inflammation referred to as “inflamm-aging” (139, 140), which is associated with increased levels of oxygen radicals, IL-15, TNFα and IFNγ (141, 142).
Taken together these data suggest that CD4+ Treg as well as CD8+ Treg accumulate in aged individuals. Dysregulation of immune homeostasis is involved in the development of different diseases in old age and a connection with increased numbers of Treg has been demonstrated for several conditions (Figure 1).
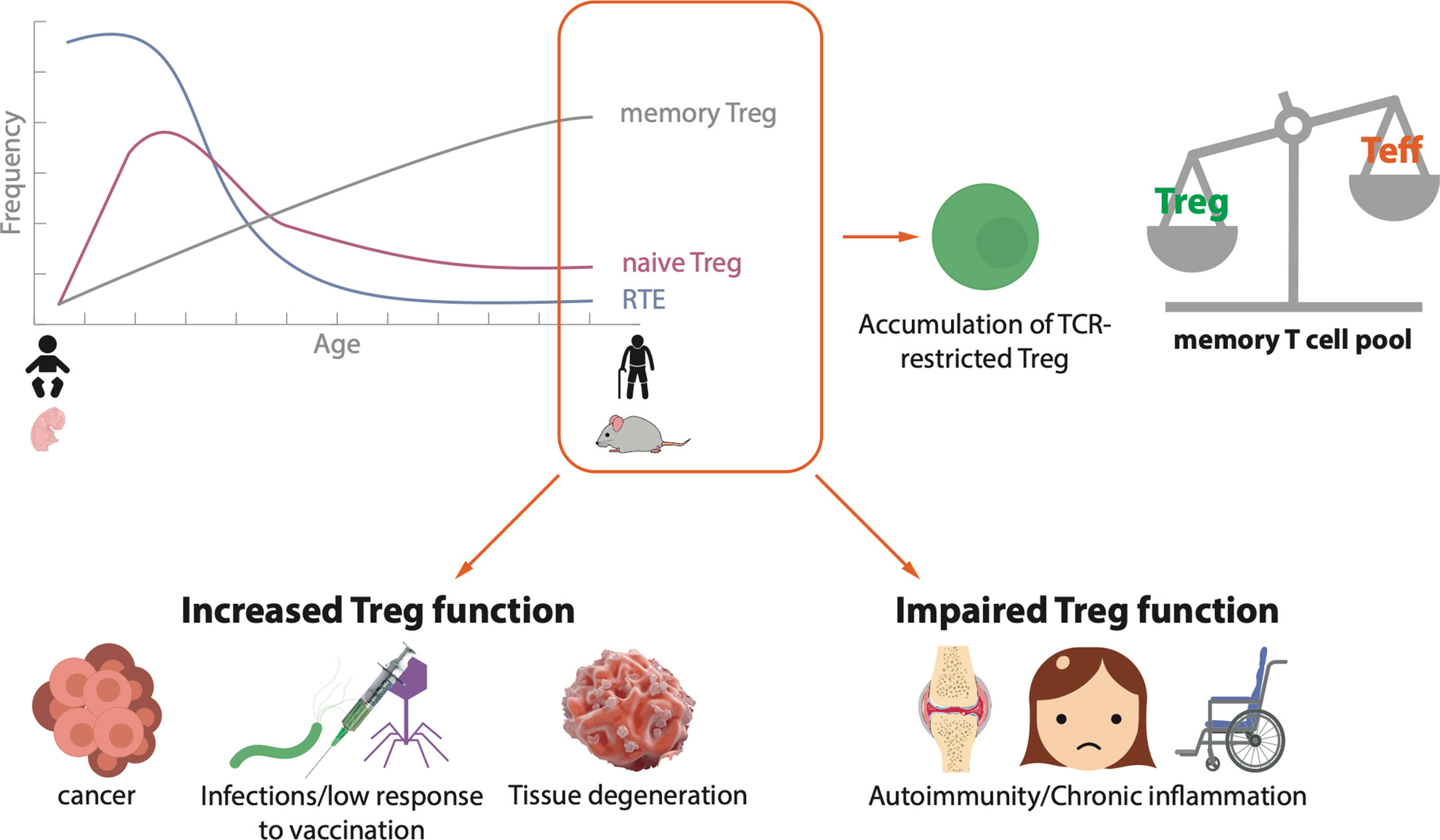
Figure 1 Schematic landscape of Treg in the elderly. During human and mouse aging, there is a decreased efflux of recent thymic emigrants (RTE) to the periphery, a reduction of naïve Treg and an accumulation of antigen experienced memory Treg. As a consequence, TCR-restricted Treg accumulate and the pool of memory T cells is skewed towards memory Treg over effector/memory T cells. This imbalance on T cell homeostasis is related to the development of different diseases. In old age, an increase in Treg function may lead to the progression of tumors, chronic infections or tissue degeneration, whereas an impaired Treg function may cause autoimmunity and/or chronic inflammation.
Discussion
The findings discussed above show that the field of regulatory cells is in continuous expansion. The more we try to define it, the more complex becomes the diversity of different cells (T, B, and NKT cells) that might be involved. Initially described as suppressor cells, Treg have demonstrated to be able to regulate several processes with the final aim of preserving immune homeostasis. To date, there are numerous types of Treg described in mouse and human (Table 1) and a lack of consensus to establish the right markers to define these populations make of their characterization a matter of controversy. The promiscuity of Foxp3 expression as a bona fide marker of Treg is a good starting point to discuss the huge diversity of Treg identified so far. There is not a single marker distinct for Treg but a combination of some of them (Foxp3, CD25; GITR; CTLA-4, IL-10, etc) together with their suppressive activity could help to identify this elusive type of cells. Furthermore, most of the studies on human Treg are based on circulating cells in the blood, which may not represent the real landscape in a given tissue.
Treg cells are extremely versatile. They exert various effector functions and use a number of molecular mechanisms depending on the tissue and the health or disease context. Dysregulation of Treg function in one or another direction can unchain a huge variety of ailments (Figure 1). Indeed, modulation of Treg activity is a main target for anti-tumor therapies, treatment of autoimmunity or the avoidance of graft rejection in transplantation, for instance. The danger of targeting Treg to treat or prevent a certain disease is that the consequence could promote the development of another one. As an example, therapies for autoimmune diseases, which enhance Treg activity in order to dampen the autoreactive immune components, have the potential to increase the risk of tumor development as the suppressed immune cells might be unresponsive to malignant cells.
The level of complexity increases further when we add the factor age. In the elderly, the immune compartment is altered due to thymic involution and dysregulation of several immune processes. Most of the studies show that there is an accumulation of antigen-experienced Treg with age, but discrepancies exist regarding the hypo- or hyper-activity of these cells. This difference in Treg accumulation may not only be age-dependent but also context-dependent (Figure 1). In some cases, this results in increased responsiveness of aged individuals to Treg targeted therapies compared to young ones, as it is the case for anti-PD1 therapy in melanoma patients (143).
The vast diversity of Treg in different tissues and the lack of molecular markers to clearly define Treg subtypes makes this area of study highly complex. The clear increase in immune regulatory cells in aging is offering the scientific community plenty of opportunities to learn the context-specific roles of Treg cells in immunosenescence, advancing the concept into a more tailored tissue/disease/Treg-specific point of view.
Author Contributions
LRR, FLM, RW, and BW wrote and discussed the manuscript, and all authors agree to be accountable for the content of the work. All authors contributed to the article and approved the submitted version.
Funding
This work was supported by funds of the Austrian Science Fund (FWF W1253-B24; doctoral programme HOROS).
Conflict of Interest
The authors declare that the research was conducted in the absence of any commercial or financial relationships that could be construed as a potential conflict of interest.
Acknowledgments
We thank G. Guem for his help with graphical design.
References
1. Sakaguchi S, Powrie F, Ransohoff RM. Re-establishing immunological self-tolerance in autoimmune disease. Nat Medicine (2012) 18(1):54–8. doi: 10.1038/nm.2622
2. Hogquist KA, Bevan MJ. The nature of the peptide/MHC ligand involved in positive selection. Semin Immunol (1996) 8:63–8. doi: 10.1006/smim.1996.0009
3. Sakaguchi S, Takahashi T, Nishizuka Y. Study on cellular events in post-thymectomy autoimmune oophoritis in mice. II. Requirement of Lyt-1 cells in normal female mice for the prevention of oophoritis. J Exp Medicine (1982) 156(6):1577–86. doi: 10.1084/jem.156.6.1577
4. Fowell D, Mason D. Evidence that the T cell repertoire of normal rats contains cells with the potential to cause diabetes. Characterization of the CD4+ T cell subset that inhibits this autoimmune potential. J Exp Medicine (1993) 177(3):627–36. doi: 10.1084/jem.177.3.627
5. Sakaguchi S, Sakaguchi N, Asano M, Itoh M, Toda M. Immunologic self-tolerance maintained by activated T cells expressing IL-2 receptor alpha-chains (CD25). Breakdown of a single mechanism of self-tolerance causes various autoimmune diseases. J Immunol (Baltimore Md 1950) (1995) 155(3):1151–64.
6. Fontenot JD, Gavin MA, Rudensky AY. Foxp3 programs the development and function of CD4+CD25+ regulatory T cells. Nat Immunol (2003) 4(4):330–6. doi: 10.1038/ni904
7. Josefowicz SZ, Lu LF, Rudensky AY. Regulatory T cells: mechanisms of differentiation and function. Annu Rev Immunol (2012) 30:531–64. doi: 10.1146/annurev.immunol.25.022106.141623
8. Hsieh CS, Liang Y, Tyznik AJ, Self SG, Liggitt D, Rudensky AY. Recognition of the peripheral self by naturally arising CD25+ CD4+ T cell receptors. Immunity (2004) 21(2):267–77. doi: 10.1016/j.immuni.2004.07.009
9. Chen W, Jin W, Hardegen N, Lei KJ, Li L, Marinos N, et al. Conversion of peripheral CD4+CD25- naive T cells to CD4+CD25+ regulatory T cells by TGF-beta induction of transcription factor Foxp3. J Exp Medicine (2003) 198(12):1875–86. doi: 10.1084/jem.20030152
10. Shevach EM, Thornton AM. tTregs, pTregs, and iTregs: similarities and differences. Immunol Rev (2014) 259(1):88–102. doi: 10.1111/imr.12160
11. Kretschmer K, Apostolou I, Hawiger D, Khazaie K, Nussenzweig MC, von Boehmer H. Inducing and expanding regulatory T cell populations by foreign antigen. Nat Immunol (2005) 6(12):1219–27. doi: 10.1038/ni1265
12. Miyara M, Yoshioka Y, Kitoh A, Shima T, Wing K, Niwa A, et al. Functional delineation and differentiation dynamics of human CD4+ T cells expressing the FoxP3 transcription factor. Immunity (2009) 30(6):899–911. doi: 10.1016/j.immuni.2009.03.019
13. Wing JB, Tanaka A, Sakaguchi S. Human FOXP3(+) Regulatory T Cell Heterogeneity and Function in Autoimmunity and Cancer. Immunity (2019) 50(2):302–16. doi: 10.1016/j.immuni.2019.01.020
14. Rubtsov YP, Rasmussen JP, Chi EY, Fontenot J, Castelli L, Ye X, et al. Regulatory T cell-derived interleukin-10 limits inflammation at environmental interfaces. Immunity (2008) 28(4):546–58. doi: 10.1016/j.immuni.2008.02.017
15. Paul G, Khare V, Gasche C. Inflamed gut mucosa: downstream of interleukin-10. European. J Clin Invest (2012) 42(1):95–109. doi: 10.1111/j.1365-2362.2011.02552.x
16. Iyer SS, Cheng G. Role of interleukin 10 transcriptional regulation in inflammation and autoimmune disease. Crit Rev Immunol (2012) 32(1):23–63. doi: 10.1615/CritRevImmunol.v32.i1.30
17. Marie JC, Liggitt D, Rudensky AY. Cellular mechanisms of fatal early-onset autoimmunity in mice with the T cell-specific targeting of transforming growth factor-beta receptor. Immunity (2006) 25(3):441–54. doi: 10.1016/j.immuni.2006.07.012
18. Akkaya B, Shevach EM. Regulatory T cells: Master thieves of the immune system. Cell Immunol (2020) 355:104160. doi: 10.1016/j.cellimm.2020.104160
19. Gondek DC, Lu LF, Quezada SA, Sakaguchi S, Noelle RJ. Cutting edge: contact-mediated suppression by CD4+CD25+ regulatory cells involves a granzyme B-dependent, perforin-independent mechanism. J Immunol (Baltimore Md 1950) (2005) 174(4):1783–6. doi: 10.4049/jimmunol.174.4.1783
20. MacDonald G, Shi L, Vande Velde C, Lieberman J, Greenberg AH. Mitochondria-dependent and -independent regulation of Granzyme B-induced apoptosis. J Exp Medicine (1999) 189(1):131–44. doi: 10.1084/jem.189.1.131
21. Fontenot JD, Rasmussen JP, Gavin MA, Rudensky AY. A function for interleukin 2 in Foxp3-expressing regulatory T cells. Nat Immunol (2005) 6(11):1142–51. doi: 10.1038/ni1263
22. Sadlack B, Merz H, Schorle H, Schimpl A, Feller AC, Horak I. Ulcerative colitis-like disease in mice with a disrupted interleukin-2 gene. Cell (1993) 75(2):253–61. doi: 10.1016/0092-8674(93)80067-O
23. Suzuki H, Kundig T, Furlonger C, Wakeham A, Timms E, Matsuyama T, et al. Deregulated T cell activation and autoimmunity in mice lacking interleukin-2 receptor beta. Science (New York NY) (1995) 268(5216):1472–6. doi: 10.1126/science.7770771
24. Bachmann MF, Oxenius A. Interleukin 2: from immunostimulation to immunoregulation and back again. EMBO Rep (2007) 8(12):1142–8. doi: 10.1038/sj.embor.7401099
25. Liao W, Lin JX, Wang L, Li P, Leonard WJ. Modulation of cytokine receptors by IL-2 broadly regulates differentiation into helper T cell lineages. Nat Immunol (2011) 12(6):551–9. doi: 10.1038/ni.2030
26. Ballesteros-Tato A, León B, Graf BA, Moquin A, Adams PS, Lund FE, et al. Interleukin-2 inhibits germinal center formation by limiting T follicular helper cell differentiation. Immunity (2012) 36(5):847–56. doi: 10.1016/j.immuni.2012.02.012
27. Kalia V, Sarkar S, Subramaniam S, Haining WN, Smith KA, Ahmed R. Prolonged interleukin-2Ralpha expression on virus-specific CD8+ T cells favors terminal-effector differentiation in vivo. Immunity (2010) 32(1):91–103. doi: 10.1016/j.immuni.2009.11.010
28. Pipkin ME, Sacks JA, Cruz-Guilloty F, Lichtenheld MG, Bevan MJ, Rao A. Interleukin-2 and inflammation induce distinct transcriptional programs that promote the differentiation of effector cytolytic T cells. Immunity (2010) 32(1):79–90. doi: 10.1016/j.immuni.2009.11.012
29. Schuler PJ, Saze Z, Hong CS, Muller L, Gillespie DG, Cheng D, et al. Human CD4+ CD39+ regulatory T cells produce adenosine upon co-expression of surface CD73 or contact with CD73+ exosomes or CD73+ cells. Clin Exp Immunol (2014) 177(2):531–43. doi: 10.1111/cei.12354
30. Ohta A, Sitkovsky M. Extracellular adenosine-mediated modulation of regulatory T cells. Front Immunol (2014) 5:304. doi: 10.3389/fimmu.2014.00304
31. Allard B, Longhi MS, Robson SC, Stagg J. The ectonucleotidases CD39 and CD73: Novel checkpoint inhibitor targets. Immunol Rev (2017) 276(1):121–44. doi: 10.1111/imr.12528
32. Fassbender M, Gerlitzki B, Ullrich N, Lupp C, Klein M, Radsak MP, et al. Cyclic adenosine monophosphate and IL-10 coordinately contribute to nTreg cell-mediated suppression of dendritic cell activation. Cell Immunol (2010) 265(2):91–6. doi: 10.1016/j.cellimm.2010.07.007
33. Yan Z, Garg SK, Banerjee R. Regulatory T cells interfere with glutathione metabolism in dendritic cells and T cells. J Biol Chem (2010) 285(53):41525–32. doi: 10.1074/jbc.M110.189944
34. Akkaya B, Oya Y, Akkaya M, Al Souz J, Holstein AH, Kamenyeva O, et al. Regulatory T cells mediate specific suppression by depleting peptide-MHC class II from dendritic cells. Nat Immunol (2019) 20(2):218–31. doi: 10.1038/s41590-018-0280-2
35. Qureshi OS, Zheng Y, Nakamura K, Attridge K, Manzotti C, Schmidt EM, et al. Trans-endocytosis of CD80 and CD86: a molecular basis for the cell-extrinsic function of CTLA-4. Science (New York NY) (2011) 332(6029):600–3. doi: 10.1126/science.1202947
36. Wu D, Wong CK, Han JM, Orban PC, Huang Q, Gillies J, et al. T reg-specific insulin receptor deletion prevents diet-induced and age-associated metabolic syndrome. J Exp Medicine (2020) 217(8):e20191542. doi: 10.1084/jem.20191542
37. Almanan MA, Raynor J, Chougnet C, Salamonis N, Amarachintha S, Steinbrecher K, et al. Type 1 regulatory T cells (Tr1) homeostasis and function in aging. J Immunol (2017) 198(1 Supplement):154.10.
38. Weiner HL. Oral tolerance: immune mechanisms and treatment of autoimmune diseases. Immunol Today (1997) 18(7):335–43. doi: 10.1016/S0167-5699(97)01053-0
39. Collison LW, Chaturvedi V, Henderson AL, Giacomin PR, Guy C, Bankoti J, et al. IL-35-mediated induction of a potent regulatory T cell population. Nat Immunol (2010) 11(12):1093–101. doi: 10.1038/ni.1952
40. Chien CH, Chiang BL. Regulatory T cells induced by B cells: a novel subpopulation of regulatory T cells. J Biomed Sci (2017) 24(1):86. doi: 10.1186/s12929-017-0391-3
41. Siegmund K, Rückert B, Ouaked N, Bürgler S, Speiser A, Akdis CA, et al. Unique phenotype of human tonsillar and in vitro-induced FOXP3+CD8+ T cells. J Immunol (Baltimore Md 1950) (2009) 182(4):2124–30. doi: 10.4049/jimmunol.0802271
42. Suciu-Foca N, Manavalan JS, Scotto L, Kim-Schulze S, Galluzzo S, Naiyer AJ, et al. Molecular characterization of allospecific T suppressor and tolerogenic dendritic cells: review. Int Immunopharmacol (2005) 5(1):7–11. doi: 10.1016/j.intimp.2004.09.003
43. Ablamunits V, Bisikirska BC, Herold KC. Human Regulatory CD8+ T Cells. Ann N Y Acad Sci (2008) 1150(1):234–8. doi: 10.1196/annals.1447.000
44. Suzuki M, Jagger AL, Konya C, Shimojima Y, Pryshchep S, Goronzy JJ, et al. CD8+CD45RA+CCR7+FOXP3+ T cells with immunosuppressive properties: a novel subset of inducible human regulatory T cells. J Immunol (Baltimore Md 1950) (2012) 189(5):2118–30. doi: 10.4049/jimmunol.1200122
45. Xystrakis E, Dejean AS, Bernard I, Druet P, Liblau R, Gonzalez-Dunia D, et al. Identification of a novel natural regulatory CD8 T-cell subset and analysis of its mechanism of regulation. Blood (2004) 104(10):3294–301. doi: 10.1182/blood-2004-03-1214
46. Vuddamalay Y, Attia M, Vicente R, Pomié C, Enault G, Leobon B, et al. Mouse and human CD8(+) CD28(low) regulatory T lymphocytes differentiate in the thymus. Immunology (2016) 148(2):187–96. doi: 10.1111/imm.12600
47. Arruvito L, Payaslián F, Baz P, Podhorzer A, Billordo A, Pandolfi J, et al. Identification and clinical relevance of naturally occurring human CD8+HLA-DR+ regulatory T cells. J Immunol (Baltimore Md 1950) (2014) 193(9):4469–76. doi: 10.4049/jimmunol.1401490
48. Flippe L, Bézie S, Anegon I, Guillonneau C. Future prospects for CD8(+) regulatory T cells in immune tolerance. Immunol Rev (2019) 292(1):209–24. doi: 10.1111/imr.12812
49. Peters C, Kabelitz D, Wesch D. Regulatory functions of γδ T cells. Cell Mol Life Sci CMLS (2018) 75(12):2125–35. doi: 10.1007/s00018-018-2788-x
50. Shalev I, Schmelzle M, Robson SC, Levy G. Making sense of regulatory T cell suppressive function. Semin Immunol (2011) 23(4):282–92. doi: 10.1016/j.smim.2011.04.003
51. Sakaguchi S, Yamaguchi T, Nomura T, Ono M. Regulatory T Cells and Immune Tolerance. Cell (2008) 133(5):775–87. doi: 10.1016/j.cell.2008.05.009
52. Cantor H, Shen FW, Boyse EA. Separation of helper T cells from suppressor T cells expressing different Ly components. II. Activation by antigen: after immunization, antigen-specific suppressor and helper activities are mediated by distinct T-cell subclasses. J Exp Medicine (1976) 143(6):1391–40. doi: 10.1084/jem.143.6.1391
53. Strioga M, Pasukoniene V, Characiejus D. CD8+ CD28- and CD8+ CD57+ T cells and their role in health and disease. Immunology (2011) 134(1):17–32. doi: 10.1111/j.1365-2567.2011.03470.x
54. Yu Y, Ma X, Gong R, Zhu J, Wei L, Yao J. Recent advances in CD8(+) regulatory T cell research. Oncol Lett (2018) 15(6):8187–94. doi: 10.3892/ol.2018.8378
55. Guillonneau C, Hill M, Hubert F-X, Chiffoleau E, Hervé C, Li X-L, et al. CD40Ig treatment results in allograft acceptance mediated by CD8CD45RC T cells, IFN-gamma, and indoleamine 2,3-dioxygenase. J Clin Invest (2007) 117(4):1096–106. doi: 10.1172/JCI28801
56. Lim A, French MA, Price P. CD4+ and CD8+ T cells expressing FoxP3 in HIV-infected patients are phenotypically distinct and influenced by disease severity and antiretroviral therapy. J Acquir Immune Defic Syndr (2009) 51(3):248–57. doi: 10.1097/QAI.0b013e3181a74fad
57. Wen Z, Shimojima Y, Shirai T, Li Y, Ju J, Yang Z, et al. NADPH oxidase deficiency underlies dysfunction of aged CD8+ Tregs. J Clin Invest (2016) 126(5):1953–67. doi: 10.1172/JCI84181
58. Zimmerer JM, Ringwald BA, Elzein SM, Avila CL, Warren RT, Abdel-Rasoul M, et al. Antibody-suppressor CD8+ T Cells Require CXCR5. Transplantation (2019) 103(9):1809–20. doi: 10.1097/TP.0000000000002683
59. Chu F, Li HS, Liu X, Cao J, Ma W, Ma Y, et al. CXCR5+CD8+ T cells are a distinct functional subset with an antitumor activity. Leukemia (2019) 33(11):2640–53. doi: 10.1038/s41375-019-0464-2
60. Wei S, Kryczek I, Zou L, Daniel B, Cheng P, Mottram P, et al. Plasmacytoid dendritic cells induce CD8+ regulatory T cells in human ovarian carcinoma. Cancer Res (2005) 65(12):5020–6. doi: 10.1158/0008-5472.CAN-04-4043
61. Lukas Yani S, Keller M, Melzer FL, Weinberger B, Pangrazzi L, Sopper S, et al. CD8(+)HLADR(+) Regulatory T Cells Change With Aging: They Increase in Number, but Lose Checkpoint Inhibitory Molecules and Suppressive Function. Front Immunol (2018) 9:1201. doi: 10.3389/fimmu.2018.01201
62. Machicote A, Belén S, Baz P, Billordo LA, Fainboim L. Human CD8(+)HLA-DR(+) Regulatory T Cells, Similarly to Classical CD4(+)Foxp3(+) Cells, Suppress Immune Responses via PD-1/PD-L1 Axis. Front Immunol (2018) 9:2788–. doi: 10.3389/fimmu.2018.02788
63. Chien Y-H, Meyer C, Bonneville M. γδ T Cells: First Line of Defense and Beyond. Annu Rev Immunol (2014) 32(1):121–55. doi: 10.1146/annurev-immunol-032713-120216
64. Godfrey DI, MacDonald HR, Kronenberg M, Smyth MJ, Kaer LV. NKT cells: what’s in a name? Nat Rev Immunol (2004) 4(3):231–7. doi: 10.1038/nri1309
65. Jiang H, Chess L. An integrated view of suppressor T cell subsets in immunoregulation. J Clin Invest (2004) 114(9):1198–208. doi: 10.1172/JCI23411
66. Lui PP, Cho I, Ali N. Tissue regulatory T cells. Immunology (2020) 161(1):4–17. doi: 10.1111/imm.13208
67. Krop J, Heidt S, Claas FHJ, Eikmans M. Regulatory T Cells in Pregnancy: It Is Not All About FoxP3. Front Immunol (2020) 11:1182. doi: 10.3389/fimmu.2020.01182
68. Attias M, Al-Aubodah T, Piccirillo CA. Mechanisms of human FoxP3(+) T(reg) cell development and function in health and disease. Clin Exp Immunol (2019) 197(1):36–51. doi: 10.1111/cei.13290
69. Luu M, Steinhoff U, Visekruna A. Functional heterogeneity of gut-resident regulatory T cells. Clin Trans Immunol (2017) 6(9):e156. doi: 10.1038/cti.2017.39
70. Lathrop SK, Bloom SM, Rao SM, Nutsch K, Lio CW, Santacruz N, et al. Peripheral education of the immune system by colonic commensal microbiota. Nature (2011) 478(7368):250–4. doi: 10.1038/nature10434
71. Mohr A, Atif M, Balderas R, Gorochov G, Miyara M. The role of FOXP3(+) regulatory T cells in human autoimmune and inflammatory diseases. Clin Exp Immunol (2019) 197(1):24–35. doi: 10.1111/cei.13288
72. Brunkow ME, Jeffery EW, Hjerrild KA, Paeper B, Clark LB, Yasayko SA, et al. Disruption of a new forkhead/winged-helix protein, scurfin, results in the fatal lymphoproliferative disorder of the scurfy mouse. Nat Genet (2001) 27(1):68–73. doi: 10.1038/83784
73. Tang Q, Adams JY, Penaranda C, Melli K, Piaggio E, Sgouroudis E, et al. Central role of defective interleukin-2 production in the triggering of islet autoimmune destruction. Immunity (2008) 28(5):687–97. doi: 10.1016/j.immuni.2008.03.016
74. Samson M, Audia S, Janikashvili N, Ciudad M, Trad M, Fraszczak J, et al. Brief report: inhibition of interleukin-6 function corrects Th17/Treg cell imbalance in patients with rheumatoid arthritis. Arthritis Rheum (2012) 64(8):2499–503. doi: 10.1002/art.34477
75. Pesce B, Soto L, Sabugo F, Wurmann P, Cuchacovich M, López MN, et al. Effect of interleukin-6 receptor blockade on the balance between regulatory T cells and T helper type 17 cells in rheumatoid arthritis patients. Clin Exp Immunol (2013) 171(3):237–42. doi: 10.1111/cei.12017
76. Burzyn D, Kuswanto W, Kolodin D, Shadrach JL, Cerletti M, Jang Y, et al. A special population of regulatory T cells potentiates muscle repair. Cell (2013) 155(6):1282–95. doi: 10.1016/j.cell.2013.10.054
77. Arpaia N, Green JA, Moltedo B, Arvey A, Hemmers S, Yuan S, et al. A Distinct Function of Regulatory T Cells in Tissue Protection. Cell (2015) 162(5):1078–89. doi: 10.1016/j.cell.2015.08.021
78. Fridman WH, Pages F, Sautes-Fridman C, Galon J. The immune contexture in human tumours: impact on clinical outcome. Nat Rev Cancer (2012) 12(4):298–306. doi: 10.1038/nrc3245
79. Turnis ME, Sawant DV, Szymczak-Workman AL, Andrews LP, Delgoffe GM, Yano H, et al. Interleukin-35 Limits Anti-Tumor Immunity. Immunity (2016) 44(2):316–29. doi: 10.1016/j.immuni.2016.01.013
80. Bos PD, Plitas G, Rudra D, Lee SY, Rudensky AY. Transient regulatory T cell ablation deters oncogene-driven breast cancer and enhances radiotherapy. J Exp Med (2013) 210(11):2435–66. doi: 10.1084/jem.20130762
81. Sakaguchi S. Naturally arising CD4+ regulatory t cells for immunologic self-tolerance and negative control of immune responses. Annu Rev Immunol (2004) 22:531–62. doi: 10.1146/annurev.immunol.21.120601.141122
82. Teng MW FNS, von Scheidt B, McLaughlin N, Sparwasser T, Smyth MJ. Conditional regulatory T-cell depletion releases adaptive immunity preventing carcinogenesis and suppressing established tumor growth. Cancer Res (2010) 70(20):7800–9. doi: 10.1158/0008-5472.CAN-10-1681
83. Filaci G, Fenoglio D, Fravega M, Ansaldo G, Borgonovo G, Traverso P, et al. CD8+ CD28- T regulatory lymphocytes inhibiting T cell proliferative and cytotoxic functions infiltrate human cancers. J Immunol (Baltimore Md 1950) (2007) 179(7):4323–34. doi: 10.4049/jimmunol.179.7.4323
84. Frassanito MA, Silvestris F, Cafforio P, Dammacco F. CD8+/CD57 cells and apoptosis suppress T-cell functions in multiple myeloma. Br J Haematol (1998) 100(3):469–77. doi: 10.1046/j.1365-2141.1998.00589.x
85. Shang B, Liu Y, Jiang SJ, Liu Y. Prognostic value of tumor-infiltrating FoxP3+ regulatory T cells in cancers: a systematic review and meta-analysis. Sci Rep (2015) 5:15179. doi: 10.1038/srep15179
86. Salama P, Phillips M, Grieu F, Morris M, Zeps N, Joseph D, et al. Tumor-infiltrating FOXP3+ T regulatory cells show strong prognostic significance in colorectal cancer. J Clin Oncol (2009) 5:1527–7755 (Electronic). doi: 10.1200/JCO.2008.18.7229
87. Saito T, Nishikawa H, Wada H, Nagano Y, Sugiyama D, Atarashi K, et al. Two FOXP3+CD4+ T cell subpopulations distinctly control the prognosis of colorectal cancers. Nat Med (2016) 22(6):679–84. doi: 10.1038/nm.4086
88. Carreras J, Lopez-Guillermo A, Fox BC, Colomo L, Martinez A, Roncador G, et al. High numbers of tumor-infiltrating FOXP3-positive regulatory T cells are associated with improved overall survival in follicular lymphoma. Blood (2006) 108(9):2957–64. doi: 10.1182/blood-2006-04-018218
89. Tzankov A, Meier C, Hirschmann P, Went P, Pileri SA, Dirnhofer S. Correlation of high numbers of intratumoral FOXP3+ regulatory T cells with improved survival in germinal center-like diffuse large B-cell lymphoma, follicular lymphoma and classical Hodgkin’s lymphoma. Haematologica (2008) 93(2):193–200. doi: 10.3324/haematol.11702
90. Voo KS, Foglietta M, Percivalle E, Chu F, Nattamai D, Harline M, et al. Selective targeting of Toll-like receptors and OX40 inhibit regulatory T-cell function in follicular lymphoma. Int J Cancer (2014) 135(12):2834–46. doi: 10.1002/ijc.28937
91. Dietze KK, Zelinskyy G, Gibbert K, Schimmer S, Francois S, Myers L, et al. Transient depletion of regulatory T cells in transgenic mice reactivates virus-specific CD8+ T cells and reduces chronic retroviral set points. Proc Natl Acad Sci U S A (2011) 108(6):2420–5. doi: 10.1073/pnas.1015148108
92. Zelinskyy G, Dietze KK, Hüsecken YP, Schimmer S, Nair S, Werner T, et al. The regulatory T-cell response during acute retroviral infection is locally defined and controls the magnitude and duration of the virus-specific cytotoxic T-cell response. Blood (2009) 114(15):3199–207. doi: 10.1182/blood-2009-03-208736
93. Ahmed A, Vyakarnam A. Emerging patterns of regulatory T cell function in tuberculosis. Clin Exp Immunol (2020) 202(3):273–87. doi: 10.1111/cei.13488
94. Knuschke T, Rotan O, Bayer W, Sokolova V, Hansen W, Sparwasser T, et al. Combination of nanoparticle-based therapeutic vaccination and transient ablation of regulatory T cells enhances anti-viral immunity during chronic retroviral infection. Retrovirology (2016) 13:24–. doi: 10.1186/s12977-016-0258-9
95. De Silva NS, Klein U. Dynamics of B cells in germinal centres. Nat Rev Immunol (2015) 15:137–48. doi: 10.1038/nri3804
96. Wing JB, Lim EL, Sakaguchi S. Control of foreign Ag-specific Ab responses by Treg and Tfr. Immunol Rev (2020) 296(1):104–19. doi: 10.1111/imr.12888
97. Clement RL, Daccache J, Mohammed MT, Diallo A, Blazar BR, Kuchroo VK, et al. Follicular regulatory T cells control humoral and allergic immunity by restraining early B cell responses. Nat Immunol (2019) 20(10):1360–71. doi: 10.1038/s41590-019-0472-4
98. Agrawal A, Agrawal S, Gupta S. Role of Dendritic Cells in Inflammation and Loss of Tolerance in the Elderly. Front Immunol (2017) 8:896. doi: 10.3389/fimmu.2017.00896
99. Drew W, Wilson DV, Sapey E. Inflammation and neutrophil immunosenescence in health and disease: Targeted treatments to improve clinical outcomes in the elderly. Exp Gerontol (2018) 105:70–7. doi: 10.1016/j.exger.2017.12.020
100. Wagner A, Weinberger B. Vaccines to Prevent Infectious Diseases in the Older Population: Immunological Challenges and Future Perspectives. Front Immunol (2020) 11:717. doi: 10.3389/fimmu.2020.00717
101. Frasca D, Blomberg BB. B cell function and influenza vaccine responses in healthy aging and disease. Curr Opin Immunol (2014) 29:112–8. doi: 10.1016/j.coi.2014.05.008
102. Pangrazzi L. Weinberger B. T cells, aging and senescence. Exp Gerontol (2020) 134:110887. doi: 10.1016/j.exger.2020.110887
103. Crooke SN, Ovsyannikova IG, Poland GA, Kennedy RB. Immunosenescence and human vaccine immune responses. Immun Ageing I A (2019) 16:25. doi: 10.1186/s12979-019-0164-9
104. Pinti M, Appay V, Campisi J, Frasca D, Fülöp T, Sauce D, et al. Aging of the immune system: Focus on inflammation and vaccination. Eur J Immunol (2016) 46(10):2286–301. doi: 10.1002/eji.201546178
105. Ray D, Yung R. Immune senescence, epigenetics and autoimmunity. Clin Immunol (2018) 196:59–63. doi: 10.1016/j.clim.2018.04.002
106. Bodey B, Bodey B Jr., Siegel SE, Kaiser HE. Involution of the mammalian thymus, one of the leading regulators of aging. In Vivo (1997) 11(5):421–40.
107. Dooley J, Liston A. Molecular control over thymic involution: from cytokines and microRNA to aging and adipose tissue. Eur J Immunol (2012) 42(5):1073–9. doi: 10.1002/eji.201142305
108. Sutherland JS, Goldberg GL, Hammett MV, Uldrich AP, Berzins SP, Heng TS, et al. Activation of thymic regeneration in mice and humans following androgen blockade. J Immunol (Baltimore Md 1950) (2005) 175(4):2741–53. doi: 10.4049/jimmunol.175.4.2741
109. Gray DH, Seach N, Ueno T, Milton MK, Liston A, Lew AM, et al. Developmental kinetics, turnover, and stimulatory capacity of thymic epithelial cells. Blood (2006) 108(12):3777–85. doi: 10.1182/blood-2006-02-004531
110. Bauer ME. The Role of Stress and Adrenal Hormones in Immunosenescence. In: Bosch JA, Phillips AC, Lord JM, editors. Immunosenescence: Psychosocial and Behavioral Determinants. New York, NY: Springer New York (2013). p. 221–39.
111. Churov AV, Mamashov KY, Novitskaia AV. Homeostasis and the functional roles of CD4(+) Treg cells in aging. Immunol Lett (2020) 226:83–9. doi: 10.1016/j.imlet.2020.07.004
112. Rocamora-Reverte L, Tuzlak S, von Raffay L, Tisch M, Fiegl H, Drach M, et al. Glucocorticoid Receptor-Deficient Foxp3(+) Regulatory T Cells Fail to Control Experimental Inflammatory Bowel Disease. Front Immunol (2019) 10:472. doi: 10.3389/fimmu.2019.00472
113. Jamieson BD, Douek DC, Killian S, Hultin LE, Scripture-Adams DD, Giorgi JV, et al. Generation of functional thymocytes in the human adult. Immunity (1999) 10(5):569–75. doi: 10.1016/S1074-7613(00)80056-4
114. Hale JS, Boursalian TE, Turk GL, Fink PJ. Thymic output in aged mice. Proc Natl Acad Sci (2006) 103(22):8447–52. doi: 10.1073/pnas.0601040103
115. Ribeiro RM, Perelson AS. Determining thymic output quantitatively: using models to interpret experimental T-cell receptor excision circle (TREC) data. Immunol Rev (2007) 216:21–34. doi: 10.1111/j.1600-065X.2006.00493.x
116. Thiault N, Darrigues J, Adoue V, Gros M, Binet B, Perals C, et al. Peripheral regulatory T lymphocytes recirculating to the thymus suppress the development of their precursors. Nat Immunol (2015) 16(6):628–34. doi: 10.1038/ni.3150
117. Garg SK, Delaney C, Toubai T, Ghosh A, Reddy P, Banerjee R, et al. Aging is associated with increased regulatory T-cell function. Aging Cell (2014) 13(3):441–8. doi: 10.1111/acel.12191
118. Carpentier M, Chappert P, Kuhn C, Lalfer M, Flament H, Burlen-Defranoux O, et al. Extrathymic induction of Foxp3+ regulatory T cells declines with age in a T-cell intrinsic manner. Eur J Immunol (2013) 43(10):2598–604. doi: 10.1002/eji.201343532
119. Chougnet CA, Tripathi P, Lages CS, Raynor J, Sholl A, Fink P, et al. A major role for Bim in regulatory T cell homeostasis. J Immunol (Baltimore Md 1950) (2011) 186(1):156–63. doi: 10.4049/jimmunol.1001505
120. Tischner D, Gaggl I, Peschel I, Kaufmann M, Tuzlak S, Drach M, et al. Defective cell death signalling along the Bcl-2 regulated apoptosis pathway compromises Treg cell development and limits their functionality in mice. J Autoimmun (2012) 38(1):59–69. doi: 10.1016/j.jaut.2011.12.008
121. Nishioka T, Shimizu J, Iida R, Yamazaki S, Sakaguchi S. CD4+CD25+Foxp3+ T cells and CD4+CD25-Foxp3+ T cells in aged mice. J Immunol (Baltimore Md 1950) (2006) 176(11):6586–93. doi: 10.4049/jimmunol.176.11.6586
122. Jagger A, Shimojima Y, Goronzy JJ, Weyand CM. Regulatory T cells and the immune aging process: a mini-review. Gerontology (2014) 60(2):130–7. doi: 10.1159/000355303
123. Raynor J, Sholl A, Plas DR, Bouillet P, Chougnet CA, Hildeman DA. IL-15 Fosters Age-Driven Regulatory T Cell Accrual in the Face of Declining IL-2 Levels. Front Immunol (2013) 4:161. doi: 10.3389/fimmu.2013.00161
124. Almanan M, Raynor J, Ogunsulire I, Malyshkina A, Mukherjee S, Hummel SA, et al. IL-10-producing Tfh cells accumulate with age and link inflammation with age-related immune suppression. Sci Adv (2020) 6(31):eabb0806. doi: 10.1126/sciadv.abb0806
125. van der Geest KS, Abdulahad WH, Tete SM, Lorencetti PG, Horst G, Bos NA, et al. Aging disturbs the balance between effector and regulatory CD4+ T cells. Exp Gerontol (2014) 60:190–6. doi: 10.1016/j.exger.2014.11.005
126. Lefebvre JS, Masters AR, Hopkins JW, Haynes L. Age-related impairment of humoral response to influenza is associated with changes in antigen specific T follicular helper cell responses. Sci Rep (2016) 6:25051. doi: 10.1038/srep25051
127. Stebegg M, Bignon A, Hill DL, Silva-Cayetano A, Krueger C, Vanderleyden I, et al. Rejuvenating conventional dendritic cells and T follicular helper cell formation after vaccination. Elife (2020) 9:e52473. doi: 10.7554/eLife.52473
128. Herrero-Fernández I, Rosado-Sánchez I, Álvarez-Ríos AI, Galvá MI, De Luna-Romero M, Sanbonmatsu-Gámez S, et al. Effect of homeostatic T-cell proliferation in the vaccine responsiveness against influenza in elderly people. Immun Ageing I A (2019) 16:14. doi: 10.1186/s12979-019-0154-y
129. Wen Z, Wang X, Dong K, Zhang H, Bu Z, Ye L, et al. Blockage of regulatory T cells augments induction of protective immune responses by influenza virus-like particles in aged mice. Microbes Infection (2017) 19(12):626–34. doi: 10.1016/j.micinf.2017.08.013
130. Goldeck D, Theeten H, Hassouneh F, Oettinger L, Wistuba-Hamprecht K, Cools N, et al. Frequencies of peripheral immune cells in older adults following seasonal influenza vaccination with an adjuvanted vaccine. Vaccine (2017) 35(34):4330–8. doi: 10.1016/j.vaccine.2017.06.082
131. van der Veeken J, Gonzalez AJ, Cho H, Arvey A, Hemmers S, Leslie CS, et al. Memory of Inflammation in Regulatory T Cells. Cell (2016) 166(4):977–90. doi: 10.1016/j.cell.2016.07.006
132. Czesnikiewicz-Guzik M, Lee W-W, Cui D, Hiruma Y, Lamar DL, Yang Z-Z, et al. T cell subset-specific susceptibility to aging. Clin Immunol (2008) 127(1):107–18. doi: 10.1016/j.clim.2007.12.002
133. Yamaguchi T, Wing JB, Sakaguchi S. Two modes of immune suppression by Foxp3+ regulatory T cells under inflammatory or non-inflammatory conditions. Semin Immunol (2011) 23(6):424–30. doi: 10.1016/j.smim.2011.10.002
134. Sun L, Hurez VJ, Thibodeaux SR, Kious MJ, Liu A, Lin P, et al. Aged regulatory T cells protect from autoimmune inflammation despite reduced STAT3 activation and decreased constraint of IL-17 producing T cells. Aging Cell (2012) 11(3):509–19. doi: 10.1111/j.1474-9726.2012.00812.x
135. Nikolich-Žugich J, Li G, Uhrlaub JL, Renkema KR, Smithey MJ. Age-related changes in CD8 T cell homeostasis and immunity to infection. Semin Immunol (2012) 24(5):356–64. doi: 10.1016/j.smim.2012.04.009
136. Fagnoni FF, Vescovini R, Mazzola M, Bologna G, Nigro E, Lavagetto G, et al. Expansion of cytotoxic CD8+ CD28- T cells in healthy ageing people, including centenarians. Immunology (1996) 88(4):501–7. doi: 10.1046/j.1365-2567.1996.d01-689.x
137. Simone R, Zicca A, Saverino D. The frequency of regulatory CD3+CD8+CD28–CD25+ T lymphocytes in human peripheral blood increases with age. J Leukocyte Biol (2008) 84(6):1454–61. doi: 10.1189/jlb.0907627
138. Motegi A, Kinoshita M, Inatsu A, Habu Y, Saitoh D, Seki S. IL-15-induced CD8+CD122+ T cells increase antibacterial and anti-tumor immune responses: implications for immune function in aged mice. J Leukocyte Biol (2008) 84(4):1047–56. doi: 10.1189/jlb.0807530
139. Franceschi C, Bonafè M, Valensin S, Olivieri F, De Luca M, Ottaviani E, et al. Inflamm-aging. An evolutionary perspective on immunosenescence. Ann N Y Acad Sci (2000) 908:244–54. doi: 10.1111/j.1749-6632.2000.tb06651.x
140. Franceschi C, Campisi J. Chronic inflammation (inflammaging) and its potential contribution to age-associated diseases. J Gerontol A Biol Sci Med Sci (2014) 69(Suppl 1):S4–9. doi: 10.1093/gerona/glu057
141. Pangrazzi L, Meryk A, Naismith E, Koziel R, Lair J, Krismer M, et al. “Inflamm-aging” influences immune cell survival factors in human bone marrow. Eur J Immunol (2017) 47(3):481–92. doi: 10.1002/eji.201646570
142. Pangrazzi L, Naismith E, Meryk A, Keller M, Jenewein B, Trieb K, et al. Increased IL-15 Production and Accumulation of Highly Differentiated CD8(+) Effector/Memory T Cells in the Bone Marrow of Persons with Cytomegalovirus. Front Immunol (2017) 8:715. doi: 10.3389/fimmu.2017.00715
Keywords: regulatory T cells, immune homeostasis, diversity, autoimmunity, inflammation, aging
Citation: Rocamora-Reverte L, Melzer FL, Würzner R and Weinberger B (2021) The Complex Role of Regulatory T Cells in Immunity and Aging. Front. Immunol. 11:616949. doi: 10.3389/fimmu.2020.616949
Received: 13 October 2020; Accepted: 07 December 2020;
Published: 27 January 2021.
Edited by:
Valquiria Bueno, Federal University of São Paulo, BrazilReviewed by:
Jorg Goronzy, Stanford University, United StatesDavid Hildeman, Cincinnati Children’s Hospital Medical Center, United States
Copyright © 2021 Rocamora-Reverte, Melzer, Würzner and Weinberger. This is an open-access article distributed under the terms of the Creative Commons Attribution License (CC BY). The use, distribution or reproduction in other forums is permitted, provided the original author(s) and the copyright owner(s) are credited and that the original publication in this journal is cited, in accordance with accepted academic practice. No use, distribution or reproduction is permitted which does not comply with these terms.
*Correspondence: Birgit Weinberger, birgit.weinberger@uibk.ac.at
†These authors have contributed equally to this work